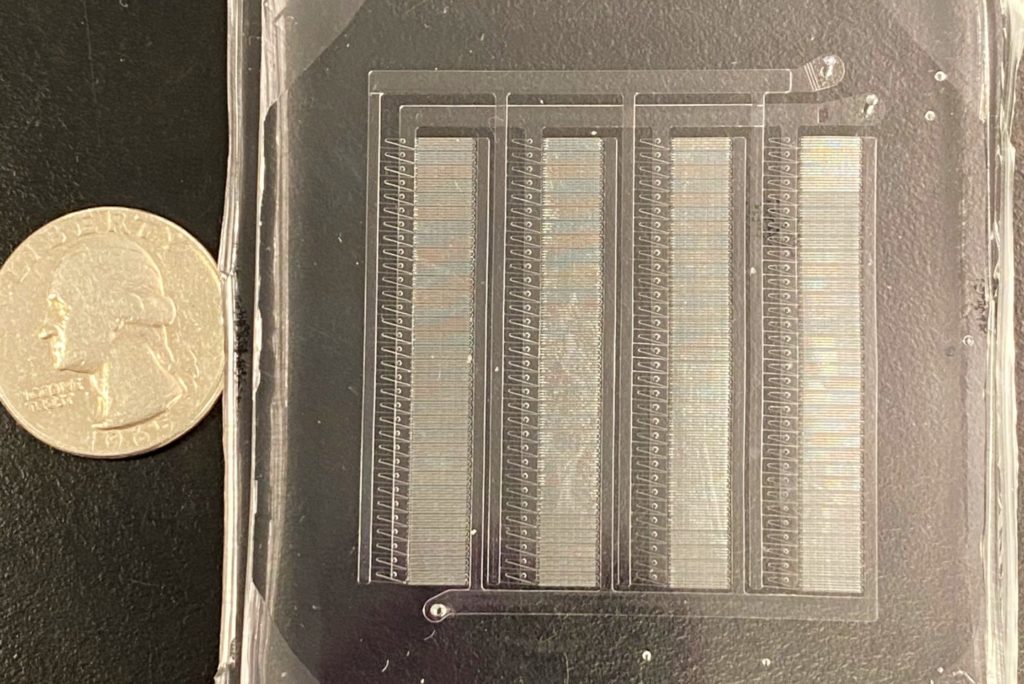
The COVID vaccines currently being deployed were developed with unprecedented speed, but the mRNA technology at work in some of them is an equally impressive success story. Because any desired mRNA sequence can be synthesized in massive quantities, one of the biggest hurdles in a variety of mRNA therapies is the ability to package those sequences into the lipid nanoparticles that deliver them into cells.
Now, thanks to manufacturing technology developed by bioengineers and medical researchers at the University of Pennsylvania, a hundred-fold increase in current microfluidic production rates may soon be possible.
The researchers’ advance stems from their design of a proof-of-concept microfluidic device containing 128 mixing channels working in parallel. The channels mix a precise amount of lipid and mRNA, essentially crafting individual lipid nanoparticles on a miniaturized assembly line.
This increased speed may not be the only benefit; more precisely controlling the nanoparticles’ size could make treatments more effective. The researchers tested the lipid nanoparticles produced by their device in a mouse study, showing they could deliver therapeutic RNA sequences with four-to-five times greater activity than those made by conventional methods.
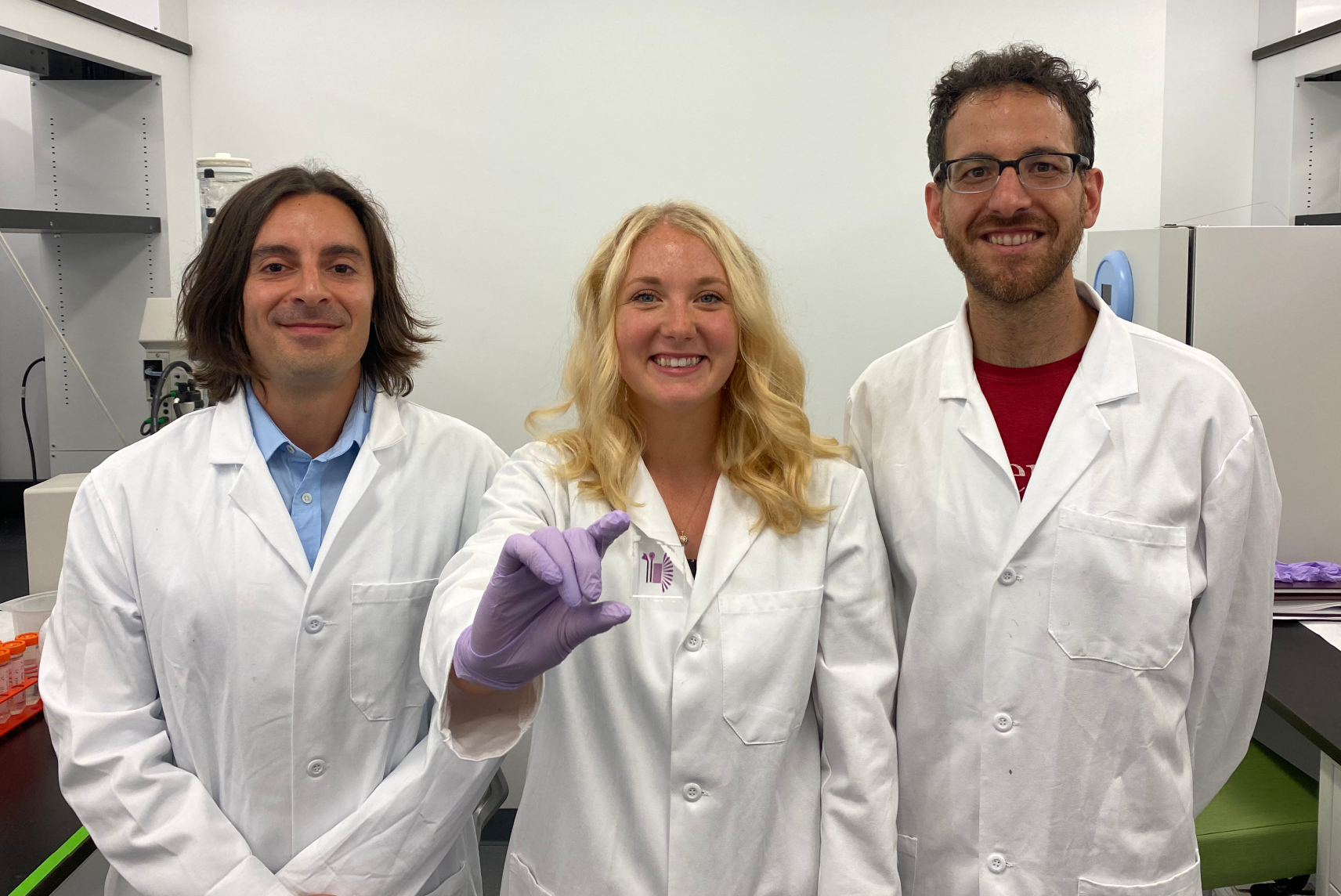
The study was led by Michael Mitchell, Skirkanich Assistant Professor of Innovation in Penn Engineering’s Department of Bioengineering, and David Issadore, Associate Professor in Penn Engineering’s Department of Bioengineering, along with Sarah Shepherd, a doctoral student in both of their labs. Rakan El-Mayta, a research engineer in Mitchell’s lab, and Sagar Yadavali, a postdoctoral researcher in Issadore’s lab, also contributed to the study.
They collaborated with several researchers at Penn’s Perelman School of Medicine: postdoctoral researcher Mohamad-Gabriel Alameh, Lili Wang, Research Associate Professor of Medicine, James M. Wilson, Rose H. Weiss Orphan Disease Center Director’s Professor in the Department of Medicine, Claude Warzecha, a senior research investigator in Wilson’s lab, and Drew Weissman, Professor of Medicine and one of the original developers of the technology behind mRNA vaccines.
It was published in the journal Nano Letters.
“We believe that this microfluidic technology has the potential to not only play a key role in the formulation of current COVID vaccines,” says Mitchell, “but also to potentially address the immense need ahead of us as mRNA technology expands into additional classes of therapeutics.”
Existing manufacturing techniques for mRNA-based vaccines use computer-controlled pumps and syringes to carefully mix two solutions: one containing the desired therapeutic mRNA and other with the oily lipids that will encapsulate them. The correct timing and ratios are key for producing usable nanoparticles, as those factors ultimately determine the nanoparticles’ size and ability to encapsulate mRNA.
With time of the essence, COVID vaccine producers opted for these tried-and-true techniques, rather than risking delays from previously unproven production technologies.
“If we don’t have the correct mixing time or ratios,” says Shepherd, “the variability in lipid nanoparticle structure will hinder its ability to survive the journey into their target cells. While we have gotten very good at determining the ideal composition for a nanoparticle, we still need to develop new production methods to formulate them rapidly and consistently.”
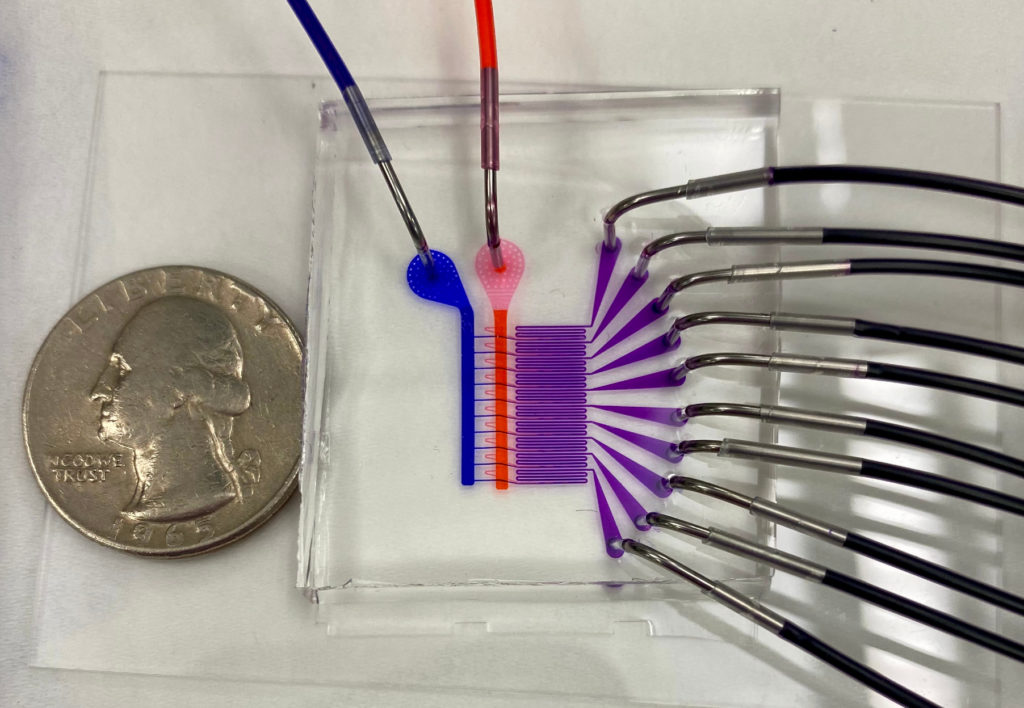
Shepherd, who works in both Mitchell’s and Issadore’s labs, was in a perfect position to spearhead a study that addressed both sides of this problem. Mitchell’s lab utilizes materials science, chemistry and computational tools to design novel biomaterials that can precisely deliver therapeutics — such as lipid nanoparticles — while Issadore’s combines elements of microelectronics, microfluidics, nanomaterials and machine learning to design microfluidic chips that are capable of manufacturing them.
“Our lab is increasingly interested in using microchips to generate precise drug formulations for the pharmaceutical industry,” Issadore says. “There’s been a tremendous amount of promise in this technology, but the successful translation to real-world applications has been rare. This is primarily due to the fundamental physics governing the flow of fluids confined within these chips’ micro- and nanoscale channels. It means that their throughput tends to be as much as a million times slower than what is necessary for commercial and clinical applications.”
Working with Mitchell’s lab, as well as with other collaborators such as Daeyeon Lee’s group, the researchers have recently developed a new microfluidics approach to address this fundamental challenge. This platform technology, called Very Large Scale Microfluidic Integration (VLSMI), allows tens of thousands of microfluidic units to be incorporated into a single three-dimensionally etched silicon-and-glass wafer.
These parallelized mixing channels allow VLSMI to have the potential to achieve the liter-per-hour production rates necessary for vaccine manufacture. Flow resistors ensure that every mixing channel receives the same flow conditions and ratio of lipids and RNAs across the device, producing the uniform nanoparticles critical for vaccine and therapeutic applications.
“Our lab has previously utilized a custom-designed microfluidic mixing technology to formulate lipid nanoparticles for mRNA therapeutics and vaccines,” Mitchell says. “However, a limitation to our in-house device was the scale of the lipid nanoparticles that we could produce. We could make enough lipid nanoparticles to dose small animals, but not larger animals and humans. The VLSMI approach was very attractive to us from early on, as we could essentially integrate our own technology into this approach so that we could operate 128 of our own mixers in parallel.”
Once the team had designed a VLSMI device capable of mass-producing RNA-carrying lipid nanoparticles, they needed to test how effective they were. Collaborating with their colleagues in Penn Medicine, they conducted studies in mice using two different types of RNA sequences, produced either by conventional mixing or their VLSMI method. The first, designed to suppress the production of a liver protein with a small interfering RNA (siRNA) sequence, showed a four-fold increase in the desired gene silencing with the VLSMI nanoparticles. The second, designed to produce a fluorescent marker protein with an mRNA sequence, showed a five-fold increase compared to conventional mixing.
These results show that VLSMI is a viable method for making lipid nanoparticles effective for use in siRNA- and mRNA-based vaccines and therapies, but the technique will need to continue to grow to meet the coming demand.
“COVID vaccines are only the beginning of the use of mRNA technology in the clinic,” Mitchell says. “The development of these vaccines will pave the way for a new wave of mRNA gene editing and protein replacement therapeutics that will revolutionize medicine. This will require the scaleup of formulating mRNA in lipid nanoparticles to unprecedented levels. We look forward to expanding upon this proof-of-concept technology with industry partners to develop scalable mRNA lipid nanoparticle therapeutics and vaccines.”
The research was supported by the U.S. National Institutes of Health Director’s New Innovator Award (DP2 TR002776), the Burroughs Wellcome Fund Career Award at the Scientific Interface, a grant by the American Cancer Society (129784-IRG-16-188-38-IRG), grants by the National Institutes of Health (NCI R01 CA241661, NCI R37 CA244911, NIDDK R01 DK123049, R33 CA206907, R21-EB023989, RM1 HG010023, R21 MH118170, R61 AI147406), the Paul G. Allen Family Foundation (Reconstructing Concussion), the Department of Defense (W81XWH1920002), the Pennsylvania Department of Health (4100077083), a National Science Foundation Graduate Research Fellowship Award (1845298), and a private funding source. This work was carried out in part at the Singh Center for Nanotechnology, which is supported by the NSF National Nanotechnology Coordinated Infrastructure Program under grant NNCI-1542153.
Issadore also has appointments in Penn Engineering’s Departments of Electrical and Systems Engineering and in Chemical and Biomolecular Engineering.