Vivek Shenoy: Mathematical Models for the Mechanical Body
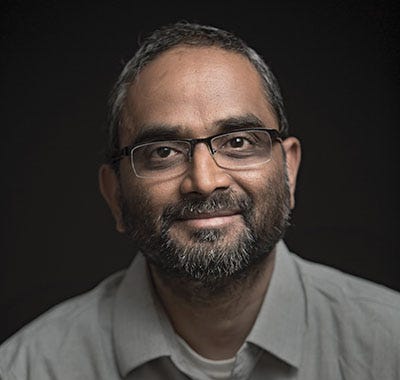
While they can seem imperfect on the surface, our bodies are in fact finely tuned machines. Joint surfaces glide effortlessly across one another. Tendons and muscles work together to control our movements, letting us run laps, hold conversations, scarf down cheesesteaks and play piano. This complex collection of biological levers, springs and pulleys is enough to keep a mechanical engineer busy for decades.
Vivek Shenoy knows this firsthand. He isn’t interested in just joints and tendons; instead he’s homing in on microscopic structures within the body and wants to understand how they shape our tissues at a basic level. A professor of Materials Science and Engineering, with secondary appointments in Mechanical Engineering and Bioengineering, Shenoy is also the co-founder and director of the $24 million NSF Science and Technology Center for Engineering Mechanobiology, where he aims to describe basic biological processes using mathematical tools.
From soft neurons to tough muscle, he notes, every cell contains a web of tiny filaments inside it. These strands, made of sugars called polysaccharides, support the cell’s contents and give it a specific structure. Webs of similar filaments also exist outside of cells, binding them to one another and fusing them into larger tissues. The properties of these fibrous “scaffolds” determine the physical qualities of our tissue. If the strands are thin and loose, they’ll make tissue that’s flaccid; if they’re tightly packed, it will be tough and firm. In addition to controlling a tissue’s physical properties, however, these scaffolds may have a much deeper impact on cells. Shenoy believes they may even affect DNA.
STRETCHING CELLS
“Let’s say a cell is suspended in a really stiff scaffold. That exerts a certain force on the entire cell, and squeezes its nucleus, which can in turn change how genes are expressed,” Shenoy says. “Some genes will be newly exposed, and so will be expressed more than usual. Others will be hidden, and will be expressed less frequently. You can effectively change the genetic function of a cell just by yanking on its nucleus.”
Those changes go both ways, Shenoy notes. While the stiffness of a cell’s scaffolding alters the genes expressed within it, some of those genes can in turn change the properties of the scaffold.
“It’s a constant feedback between mechanics and chemistry,” he says. “As stiffness or tension increases outside of a cell, it expresses genes that can then affect the tension of the cytoskeleton.” If the cytoskeleton relaxes, the cell deforms, changing the tension of filaments connected to its exterior.
Think of it a bit like a beach ball suspended in the middle of a room. Long threads connect it to the floor, ceiling and walls. When the ball is fully inflated, all those threads have equal tension on them. But if the ball loses air, some of the strands go slack. The same interplay is at work within human tissue.
THE MECHANICS OF CANCER
This sort of physical-chemical feedback could have big implications for diseases like cancer. For a tumor cell to spread throughout the body, it has to work its way through a thicket of connective tissue, dive into blood vessels and plant its flag somewhere new. It’s no small feat.
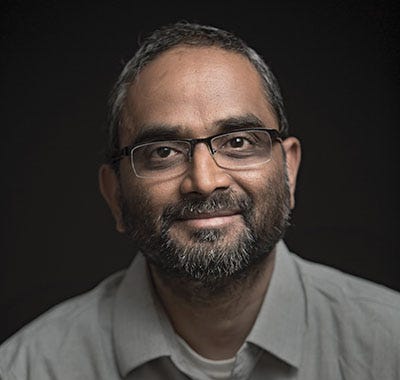
In his lab, however, Shenoy found one way that cancer cells get around this challenge. Instead of forcing their way through tough extracellular fibers, they simply change those fibers’ properties. As the cells attach to each individual strand, they can break the links that hold the fibers together, weakening connective tissue. Like explorers trailblazing through dense jungle, they can then make their way through the resulting holes.
Shenoy tested this in the lab by creating a gel that simulated human tissue. Within 12 hours of seeding it with cancer cells, he says, its previously uniform density had totally changed. In some areas, it stayed firm and resilient, but in others, it softened dramatically, letting cancer cells spread at will.
LEARNING NEW DISCIPLINES
Currently, Shenoy and his lab are working on better understanding this process. If they can chip away at the basic science behind it, he reasons, it might be possible to one day develop drugs that stop cancers from metastasizing in the first place.
It’ll take more than just a mechanical engineering approach. Teasing out the connections between mechanics and biochemistry requires a number of different disciplines, including biology, medicine, engineering and physics. That’s the impetus behind Penn’s Center for Engineering Mechanobiology.
“The core mission of our Center is to expand the capability of graduate students and postdocs across the physical and biological sciences,” says Jim McGonigle, managing director. “We have some students who are traditional biologists, with no engineering and little computational background, and others that are engineers with no experience with living cells and tissue. Our goal is to give them all the skills to interact in a meaningful way and learn from one another.”
To that end, Shenoy hosts an annual “Boot Camp” for new students, where he starts to fill the holes in their knowledge. “We start with the fundamentals, like understanding force and balance. Then, gradually, you add more and more mathematical concepts. Soon you start to realize that you can use them to write equations describing biological systems,” Shenoy says.
Shenoy and his team ultimately want to create mathematical models of biology on a few different microscopic scales, ranging from individual molecules and genes to entire tissues.
Right now, it’s an exercise in basic science, he says, but it could someday be used to engineer new tissue or build organs-on-chips, high-tech devices that let researchers predict how new drugs affect human tissues. The work could even help scientists to better understand how stem cells and embryos form. But first, Shenoy states, he wants to change the way students think about the fledgling field.
“It’s not limited to biology. I want to give students the tools to apply theory and mathematical concepts to any difficult problem. There are only so many concepts out there in the physical sciences, but the same concept can be applied in many different ways,” he says. “We’re always trying to solve puzzles from different areas. That’s the most attractive part of the job.”