Penn Engineers Solve the Paradox of Why Tissue Gets Stiffer When Compressed
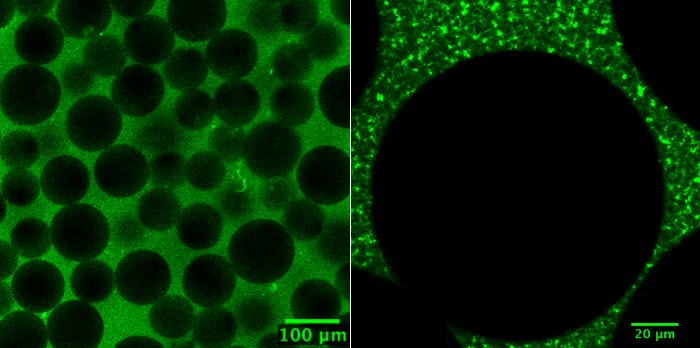
Tissue gets stiffer when it’s compressed. That property can become even more pronounced with injury or disease, which is why doctors palpate tissue as part of a diagnosis, such as when they check for lumps in a cancer screening. That stiffening response is a long-standing biomedical paradox, however: tissue consists of cells within a complex network of fibers, and common sense dictates that when you push the ends of a string together, it loosens tension, rather than increasing it.
Now, in a study published in Nature, University of Pennsylvania’s School of Engineering and Applied Science researchers have solved this mystery by better understanding the mechanical interplay between that fiber network and the cells it contains.
The researchers found that when tissue is compressed, the cells inside expand laterally, pulling on attached fibers and putting more overall tension on the network. Targeting the proteins that connect cells to the surrounding fiber network might therefore be the optimal way of reducing overall tissue stiffness, a goal in medical treatments for everything from cancer to obesity.
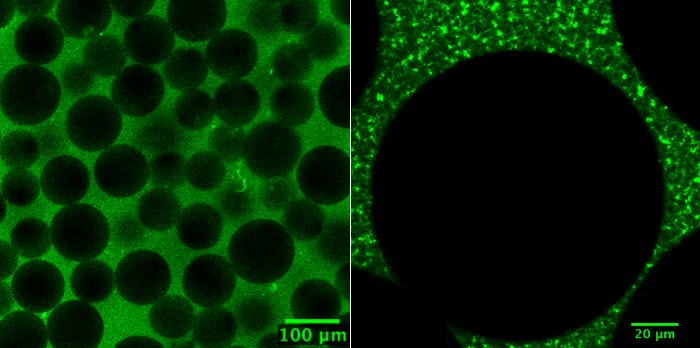
The study was led by Paul Janmey, Professor in the Perelman School of Medicine’s Department of Physiology and in Penn Engineering’s Department of Bioengineering, and Vivek Shenoy, Eduardo D. Glandt President’s Distinguished Professor in Penn Engineering’s Department of Materials Science and Engineering, along with Anne van Oosten and Xingyu Chen, graduate students in Janmey’s and Shenoy’s labs. Van Oosten is now a postdoctoral fellow at Leiden University in The Netherlands.
Shenoy is Director of Penn’s Center for Engineering Mechanobiology, which studies how physical forces influence the behavior of biological systems; Janmey is the co-director of one of the Center’s working groups, organized around the question, “How do cells adapt to and change their mechanical environment?”
Together, they have been interested in solving the paradox surrounding tissue stiffness.
“The fiber networks in and around cells don’t do what the tissue does; they do the exact opposite,” Janmey says. “The assumption was that if we understood the physics of internal polymer networks we’d understand the physics of the cell, and on a larger scale, if we understood the physics of the collagen network between cells, we’d understand the physics of the tissue. But that’s just not the case.”
The researchers began their search for the fundamentals of this relationship by experimenting on reconstituted fibrin networks, essentially tissues without cells. These polymeric materials are widely used for surgical filler, and unlike natural tissue, don’t become stiffer when they’re compressed. This was the team’s first lead as to what was driving this paradoxical reaction.
“The cell is built to maintain its volume. You can squish and deform it, but it will always take up the same amount of space,” Janmey says. “Whereas these reconstituted networks just have water instead of cells. They’re like a sponge; when you squish it, the water just leaves.”
The team’s experiments involved imbuing these reconstituted fiber networks with artificial cells — balls of starch-like particles that also maintain their volume when squished. As more and more of these particles were added, the synthetic tissue started behaving more like a natural one.
“You can’t just measure the properties of the cells and the properties of the network and then weight them to predict the properties of the composite,” Shenoy says. “Tissue ends up being a very complex, non-homogeneous material that is very sensitive to small changes. We had to make a non-linear model; you can’t just take an average.”
Based on this model, the core of the tissue paradox was the changing shape of the cells.
“The fibers themselves become stiffer in tension and softer in compression. That’s easy to understand, since if I stretch a wire, it becomes taut,” Shenoy says. “But when you sit on an exercise ball, it flattens out and the equator gets larger. That’s what’s happening to the individual cells when you push down on the tissue, which means they are stretching the fibers that are attached to it in a lateral way.”
This dynamic might explain another long-standing paradox in the field of cancer biology.
“Even though most tumors, especially breast and colorectal tumors, are stiffer than healthy tissue, individual cancer cells are usually softer than healthy cells,” Janmey says. “For a long time that made absolutely no sense, because people assumed the stiffness of the tissue was related to the stiffness of the cell. This model shows that relationship is exactly backwards.”
Because cancer cells are softer, they deform more easily under compression, and thus put more tension on their surrounding fibers. This insight might be useful in directing cancer treatments, which have historically been focused on softening either the cancerous cells or their surrounding fiber networks as a way of slowing tumor growth.
“Our experiments show that a new idea might be to loosen the connection between the cells and the fiber matrix,” Janmey says. “That wouldn’t have been obvious before, but if you make that interface floppier, you don’t need to soften either one.”
The relationships outlined in the researchers’ study could lend itself to an even more fundamental understanding of why tissue stiffness is associated with disease. The interplay between cells and their surrounding fiber matrix triggers signal transduction pathways that can have wide-ranging effects. Other research in the field suggests that stiffer environments are more conducive to cellular damage and mutation, and that the stiffer fat cells that go along with obesity can trigger various inflammatory responses.
“On a more basic level,” Shenoy says, “this is why if you sit for an hour, you feel stiff! The compressive stress is triggering signaling pathways at the cellular level.”
LiKang Chin, Katrina Cruz, Alison E. Patteson and Katarzyna Pogoda, all members of Janmey’s lab, also contributed to the study.
This work was supported by the National Institutes of Health through grants R01GM09697, GM111942, U54-CA193417 and EB017753; the National Science Foundation through grants DMR-1120901, DMR-1720530, R01CA232256 and U01CA202177; the NSF Center for Engineering Mechanobiology through grant CMMI-154857; by a Fulbright Science and Technology Award; by Prins Bernhard Cultuurfonds-Kuitse Fonds; and partially supported by the National Science Center, Poland under grant number UMO2017/26/D/ST4/00997 and the Polish-American Fulbright Commission.