Unique electrical properties in quantum materials can be controlled using light
New research on Weyl semimetals, a class of quantum materials, unlocks unique quantum properties that can be used to create light-controlled electronic devices in the future.
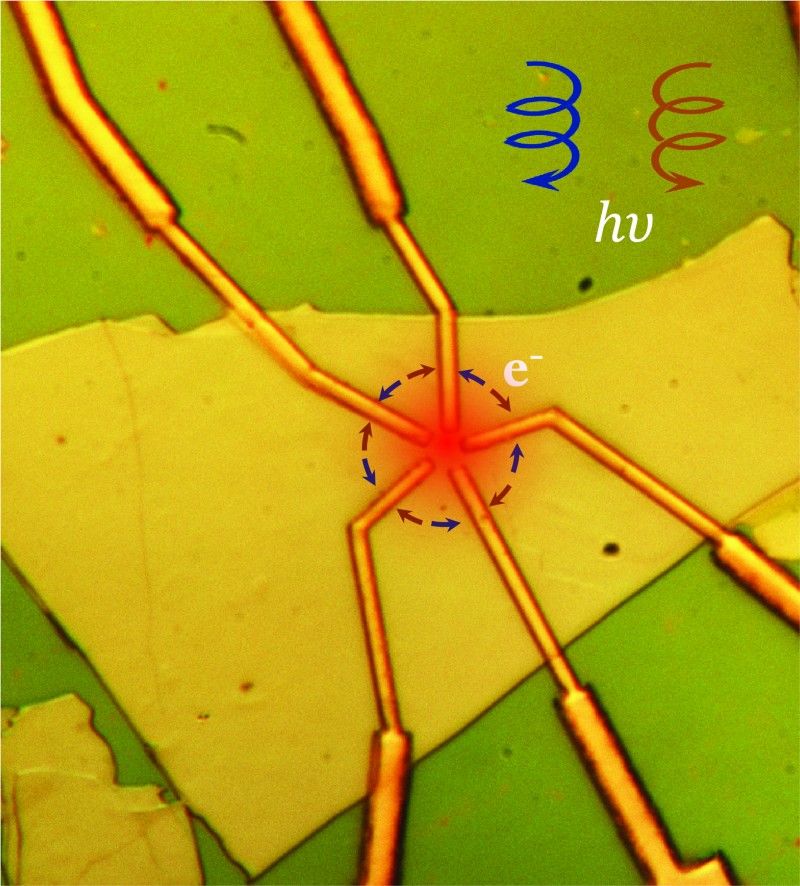
By Erica K. Brockmeier
Insights from quantum physics have allowed engineers to incorporate components used in circuit boards, optical fibers, and control systems in new applications ranging from smartphones to advanced microprocessors. But, even with significant progress made in recent years, researchers are still looking for new and better ways to control the uniquely powerful electronic properties of quantum materials.
A new study from Penn researchers found that Weyl semimetals, a class of quantum materials, have bulk quantum states whose electrical properties can be controlled using light. The project was led by Ritesh Agarwal and graduate student Zhurun Ji in the School of Engineering and Applied Science in collaboration with Charles Kane, Eugene Mele, and Andrew M. Rappe in the School of Arts and Sciences, along with Zheng Liu from Nanyang Technological University. Penn’s Zachariah Addison, Gerui Liu, Wenjing Liu, and Heng Gao, and Nanyang’s Peng Yu, also contributed to the work. Their findings were published in Nature Materials.
A hint of these unconventional photogalvanic properties, or the ability to generate electric current using light, was first reported by Agarwal in silicon. His group was able to control the movement of electrical current by changing the chirality, or the inherent symmetry of the arrangement of silicon atoms, on the surface of the material.
“At that time, we were also trying to understand the properties of topological insulators, but we could not prove that what we were seeing was coming from those unique surface states,” Agarwal explains.
Then, while conducting new experiments on Weyl semimetals, where the unique quantum states exist in the bulk of the material, Agarwal and Ji got results that didn’t match any theories that could explain how the electrical field was moving when activated by light. Instead of the electrical current flowing in a single direction, the current moved around the semimetal in a swirling circular pattern.
Agarwal and Ji turned to Kane and Mele to help develop a new theoretical framework that could explain what they were seeing. After conducting new, extremely thorough experiments to iteratively eliminate all other possible explanations, the physicists were able to narrow the possible explanations to a single theory related to the structure of the light beam.
“When you shine light on matter, it’s natural to think about a beam of light as laterally uniform,” says Mele. “What made these experiments work is that the beam has a boundary, and what made the current circulate had to do with its behavior at the edge of the beam.”
Using this new theoretical framework, and incorporating Rappe’s insights on the electron energy levels inside the material, Ji was able to confirm the unique circular movements of the electrical current. The scientists also found that the current’s direction could be controlled by changing the light beam’s structure, such as changing the direction of its polarization or the frequency of the photons.
“Previously, when people did optoelectronic measurements, they always assume that light is a plane wave. But we broke that limitation and demonstrated that not only light polarization but also the spatial dispersion of light can affect the light-matter interaction process,” says Ji.
This work allows researchers to not only better observe quantum phenomena, but it provides a way to engineer and control unique quantum properties simply by changing light beam patterns. “The idea that the modulation of light’s polarization and intensity can change how an electrical charge is transported could be powerful design idea,” says Mele.
Future development of “photonic” and “spintronic” materials that transfer digitized information based on the spin of photons or electrons respectively is also made possible thanks to these results. Agarwal hopes to expand this work to include other optical beam patterns, such as “twisted light,” which could be used to create new quantum computing materials that allow more information to be encoded onto a single photon of light.
“With quantum computing, all platforms are light-based, so it’s the photon which is the carrier of quantum information. If we can configure our detectors on a chip, everything can be integrated, and we can read out the state of the photon directly,” Agarwal says.
Agarwal and Mele emphasize the “heroic” effort made by Ji, including an additional year’s measurements made while running an entirely new set of experiments that were crucial to the interpretation of the study. “I’ve rarely seen a graduate student faced with that challenge who was able not only to rise to it but to master it. She had the initiative to do something new, and she got it done,” says Mele.
This research was supported by Office of Naval Research (Grant N00014–17–1–2661), U.S. Army Research Office (Grant W911NF- 17–1–0436), U.S. Department of Energy (grants DE FG02 84ER45118 and DE-FG02–07ER46431), and a National Science Foundation Materials Research Science and Engineering Centers seed grant.