When do materials bend and when do they break? For Ottman Tertuliano, AMA Family Assistant Professor in Mechanical Engineering and Applied Mechanics (MEAM), this question is one he normally investigates in human bones. But in a new study with Robert Carpick, John Henry Towne Professor in MEAM, Tertuliano turns to graphene, a tough, flexible and lightweight material extracted from graphite and made up of pure carbon, to answer this question.
Published in Nano Letters and led by Luc Capaldi, a MEAM Ph.D. student in Tertuliano’s lab with support from Carpick’s current and former team members Li Yuan, Cangyu Qu and Daniel Sanchez, the study describes a new, 3D auto-kirigami deformation of graphene, one of the strongest materials known to science, and how the process could be used to transform 2D graphene into 3D structures, paving the way for more diverse applications of this mighty material in the third dimension.
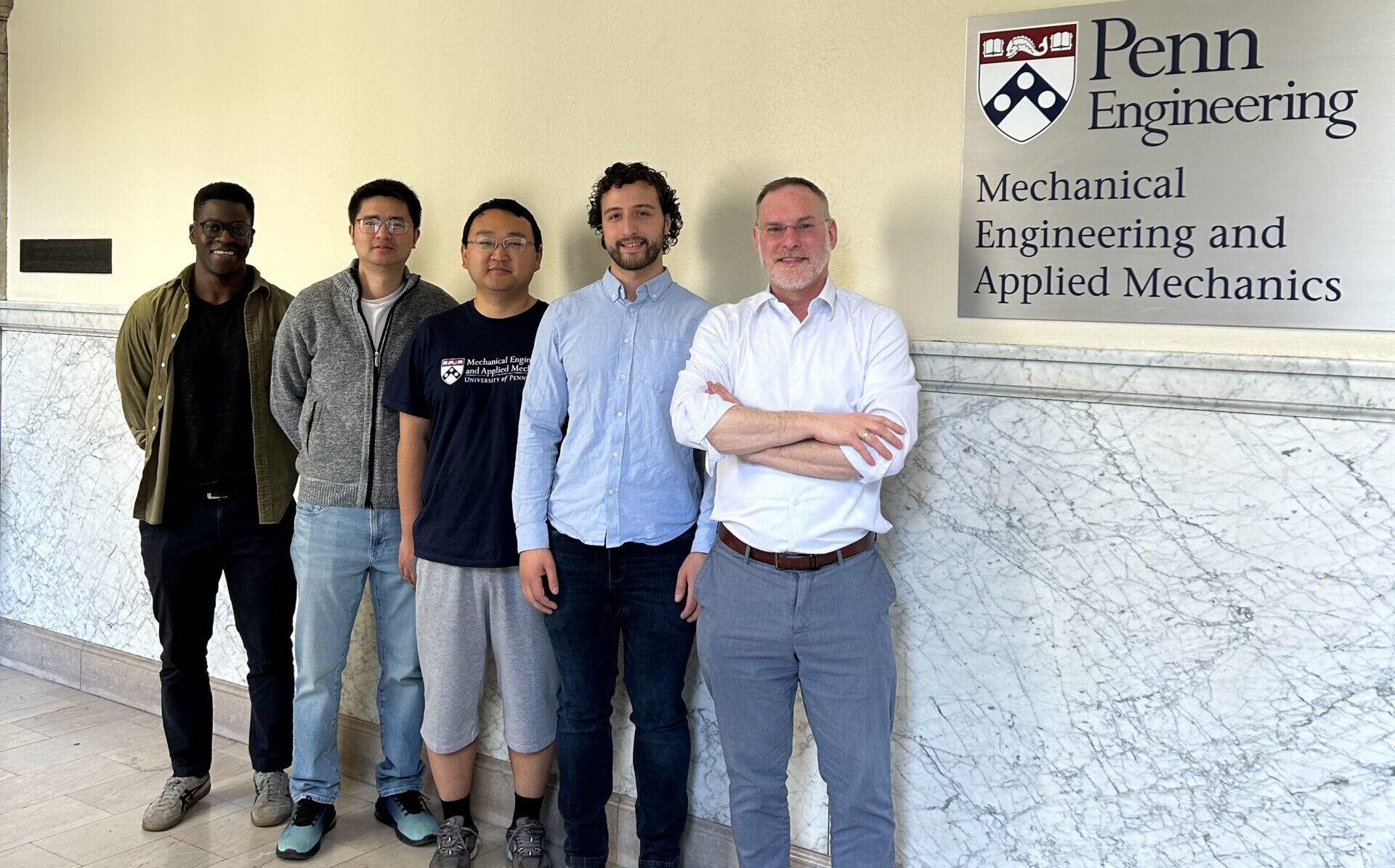
Graphene is famous in engineering circles for its suite of superpowers. As little as just a single atom thick, it’s stronger than steel, lighter than aluminum and conducts electricity better than copper. But in 2016, a paper by G.L.W. Cross at Trinity College Dublin hinted at another, more surprising property: when you indent graphene, it doesn’t just crack, it folds back onto itself and begins to tear in a predictable way. It self-organizes. Tertuliano and Carpick call it “auto-kirigami.”
“This was really intriguing,” says Tertuliano. “Because it meant the material was doing something we typically engineer from the outside. It was finding new shapes by itself.”
While others had noted this effect, the challenge remained: how could engineers see it happening, and more importantly, learn to control it?
Observing the process was only made possible through collaboration.
“My lab has been studying nanoscale friction, adhesion and mechanics for several years, including for 2D materials and layered materials like graphene and graphite,” says Carpick. “We wanted to know what happens when graphene and graphite are broken by poking them with a sharp indenter, but our method only lets us see the shape of the broken structure when the process is complete. We looked to Ottman and his lab’s nanoindenter to see what was happening in real time. When does it break? Does it change and evolve after it breaks? We couldn’t tell, but Ottman and Luc could because they can perform the experiments inside an electron microscope.”
“We had the nanoscale imaging tools Rob needed to look at this mechanism,” says Tertuliano. “We routinely use high-speed electron and X-ray microscopy to observe how bones fracture in real time. The question was, could we do the same with graphene?”
There was one issue: graphene is just too thin. At a single atomic layer, it moves and deforms faster than what current imaging tools, including Tertuliano’s in situ electron microscopy experiments, can capture.
So they changed their approach. Capaldi began experimenting with thicker graphene films, layering sheets together using a simple tape exfoliation method. The goal: make the deformation visible by increasing the dimensionality.
“We really struggled for a long time with the single layer experiment, not understanding what to optimize first,” says Capaldi. “First we optimized our instrument. Then we started using a spherical nanoindentation tip instead of a sharp one. Unfortunately, we still couldn’t see the single-layer deformation. It wasn’t until fixing everything else that we tried indenting thicker stacks of graphene. That combination of variables led to the formation of our first 3D auto-kirigami. Once I saw this distinct shape in the optical microscope, I immediately knew we had something cool.”
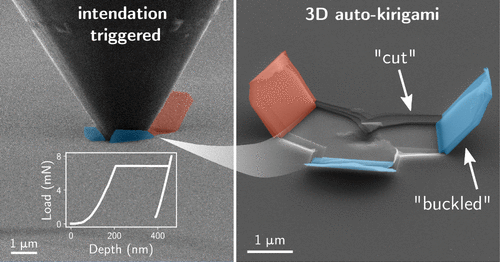
What they found was that by punching a hole through 30 graphene sheets, only about 1/1000th of the thickness of a human hair, the material transforms in ways that are not just resilient, but smart. This study uncovers the mechanics behind auto-kirigami, where thin sheets of graphene autonomously fold, fracture and rise into three-dimensional structures, all in the blink of an eye.
“When you go from one sheet to 30 or 50, the behavior changes,” explains Tertuliano. “Instead of the leaflet folding back onto itself, it stands up. It buckles. It exposes new surfaces.”
Video footage captured under electron microscopy that shows the once-flat material now forms autonomous 3D leaflets like tiny ridges or “whiskers” that point up, out and away from the original plane.
These standing structures open new electrical pathways, allowing electrons to move not just laterally, but vertically and diagonally across the sheet. Early measurements suggest that this self-folded geometry can at least double the conductivity in some directions.
While traditional material design often relies on chemistry — coatings, doping, etching — this work shows that mechanical forces alone can achieve highly precise outcomes.
“This is where mechanics takes the cake,” Tertuliano says. “You can deform a material mechanically and get it to fold or slide or stand up, all autonomously, and in milliseconds. That’s huge for scalability and efficiency.”
The implications are vast. Engineers can now imagine creating devices like flexible conductors, mechanical grippers or optical sensors that manipulate light, all from a single piece of graphene, shaped by nothing more than a carefully applied indentation.
“This paper is only a first step, but it does show that we can take a flat, layered material and make elementary structures that ‘pop up’ out of plane, like a pop-up book,” says Carpick. “This mechanical dance may offer a revolutionary way to design next-generation technologies such as small-scale robots that can be used for microsurgery or as energy harvesters that convert mechanical energy into stored electric energy. They could also be used as metamaterials, which can steer and guide light in special ways thanks to their nanoscale structuring.”
What makes this discovery even more exciting is that it’s not limited to graphene. Other van der Waals materials — 2D materials that stack via weak interlayer forces — also show similar behaviors. With different stacking combinations, it’s possible to build ultra-lightweight, thin, multifunctional devices tailored for specific tasks.
“My work on bone fracture and on graphene really asks the same thing: how do materials know when to bend, and when to break?” says Tertuliano. “In the body, bones will establish small fractures to avoid a more catastrophic break somewhere else. Other materials can work the same way. It’s about using fracture as a feature.”
As the team celebrates these foundational milestones in the research of 3D graphene auto-kirigami, future projects are already on their horizon. Next, they hope to apply the results from this study to the design and manufacturing to actualize the devices mentioned above. They will also be looking for new phenomena in 2D materials that can be triggered by cutting or indenting, including combining different 2D materials together to make heterogeneous stacks of 2D materials with unique properties and implications for additional novel electronic devices.
This work was supported by the National Science Foundation (NSF) under a Graduate Research Fellowship Program Award, the US-Ireland R&D Partnership Program Grant (CMMI-2041662), an NSF CAREER Award (2339836), an NSF grant under the American Society for Engineering Education (#EEC-2127509), and Penn’s Provost’s Postdoctoral Fellowship from the University of Pennsylvania. This work was carried out in part at the Singh Center for Nanotechnology, which is supported by the NSF National Nanotechnology Coordinated Infrastructure Program under grant NNCI-2025608.